Thank you for visiting nature.com. You are using a browser version with limited support for CSS. To obtain the best experience, we recommend you use a more up to date browser (or turn off compatibility mode in Internet Explorer). In the meantime, to ensure continued support, we are displaying the site without styles and JavaScript.
Scientific Reports volume 12, Article number: 21059 (2022 ) Cite this article Wastewater Treatment Chemicals

Iron nanoparticles have been biosynthesized by a new Aspergillus flavipes isolate. Size of biosynthesized iron nanoparticles was in a range of 32.7 and 47.6 nm, their surface charge was − 33.5 ± 5.3 and they showed semihard ferromagnetic behavior. Salt concentration, volume of added culture filtrate and pH have been optimized using response surface methodology. A significant effect for the added culture filtrate and a mutual interaction between this factor and the pH has been detected. Model validation results showed 3.3% deviation from the statistically predicted values which reflects the accuracy of the employed model. Optimization process has increased the quantity of iron in the prepared samples and the amount of produced iron nanoparticles to a fourfold. The optimized conditions have stimulated the formation of nanoparticles in a tetrahedron shape rather than the truncated tetrahedron shape without affecting their size or surface charge. The biosynthesized iron nanoparticles have recorded a good decolorization activity for methylene blue. They showed 57 ± 4.3 decolorization percent after 6 h when tested with only 0.1 mg/ml concentration. Moreover, 50 ppm concentration has exerted a detectable antifungal activity against Alternaria solani. This study represents a new competitive green synthesis method for magnetic iron nanoparticle.
Ramya M. Subramani, Robert Lotha, … Arvind Sivasubramanian
Hassan S. Hassan, Deyaa Abol-Fotouh, … Marwa F. Elkady
Sabah Ansar, Hajera Tabassum, … Roua Alsubki
Nanotechnology is a branch of science that deals with the use of materials at nanoscale. This concept was firstly introduced in 1959 by Richard Feynman and the term “nanotechnology” was firstly used by a Japanese scientist called Norio Taniguchi in 19741,2.
A nanoparticle can be defined as a minute particle with dimensions ranging between 1 and 100 nm (10–9 m). It can be considered as a turning point between bulk material and atomic-scale material2,3 Compared to their bulk materials, nanoparticles have unique and enhanced characters due to their higher surface to volume ratio4. As a result, they show higher reactivity, higher surface potential, tunable physical/chemical properties1. In addition, they may show extraordinary thermal, optical, and electrochemical properties which give them a great value in many application fields such as the industrial, agricultural, medical and environmental fields3.
Nanoparticles can be produced by two techniques “Top-down” approach and “Bottom-up” approach2,4. In case of “Top-down” approach, nanoparticles are produced from their bulk materials utilizing physical methods such as mechanical milling, laser ablation and sonication. These techniques are time-consuming and yield non-uniformly distributed particles. On the other hand, “Bottom-up” approach builds up the nanoparticles from their molecular base utilizing chemical and biological techniques4. Chemical strategies usually involve the use of harmful compounds and release some toxic byproducts making them unfavorable methods due to the resulted environmental issues5. In biological methods, biological components are utilized for synthesis process. This method is considered as a promising technique as it is safe, eco-friendly, and economically effective. Moreover, it yields well-defined sized nanoparticles4,6.
Amongst the metallic nanomaterials, iron nanoparticles have gained a great attention due to their low toxicity, good reactivity, and magnetic behavior. Such characteristics make them one of the best competitors in nano science. Indeed, iron nanoparticles have been efficiently used in medicine, agriculture, food, paint, textile, cosmetics, and wastewater treatment fields4. Magnetic behavior of iron nanoparticles added a special significance to them in certain applications such as magnetic, electrical and biomedical applications. This magnetic behavior has a great value in directed drug delivery, labeling, magnetic separation of biological materials3 in addition to the wastewater treatment.
Plants, bacteria and fungi have been utilized in green/bio synthesis of iron nanoparticles; however fungal mediated methods can be considered as the most efficient one as fungi are potent producers of bioactive compounds which play a role in the production process of nanoparticles as reductive agents7. Moreover, fungi are easy to be handled, show fast growth rate and its cultivation and maintenance methods are costly effective6. Despite that, little studies have been performed on myco-synthesis of zero valent iron nanoparticles.
Thus, the present study has focused on the use of fungi in green synthesis of magnetic iron nanoparticles. Moreover, this study has investigated how to optimize some parameters in the production process to enhance their productivity.
Optimization process is traditionally carried out by the one factor at time approach (OFAT)8,9. In this technique one factor is studied at a time while the other factors are kept constant9. Hence, this approach is a time-consuming where the factors must be optimized one by one. Moreover, such method ignores the mutual interaction which possibly occurs between the tested factors. Therefore, quality professionals prefer to use other statistically based methods such as design of experiment (DOE) methods8,9 which can estimate both the main effect and the interaction between the tested factors9,10,11,12. Response surface methodology is one of such statistically designs that generates a response surface map and estimates how to move the process to the optimum location8. Consequently, this method has been selected to perform the employed optimization study.
Finally, the effectiveness of the produced iron nanoparticles as dye decolorizing and antifungal agent has been investigated in a trial to evaluate their applied value.
The investigated fungus has been isolated from soil sediment of Avicennia marina (Forssk.) Vierh. growing on Red Sea coast at Ras Mohammad—South Sinai—Egypt (27°44′30″ N, 34°14′05″ E) using soil dilution plate method described by Waksman13 and Kumar et al.14. This isolate has been identified by both molecular and morphological methods. Genomic DNA was extracted from the fungal mat using cetyl trimethylammonium bromide (CTAB) method15,16. ITS4 primer was used for amplification of ribosomal internal transcribed spacer (ITS). PCR product was purified and sequenced at Macrogen Company—Seoul—South Korea. The obtained DNA sequence was identified utilizing Basic Local Alignment Search Tool (BLAST) available on the website of National Center of Biotechnology Information (NCBI). Phylogenetic analysis was performed using (NCBI) and MegAlign (DNA Star) software version 5.05. The molecular identification was confirmed by studying the growth, morphological and microscopical characters of the investigated isolate.
The ability of obtained fungal isolate to synthesis iron nanoparticles extracellularly has been investigated. Two fungal discs (0.8 cm diameter using cork borer) were inoculated to 250 ml conical flasks, containing 50 ml of MGYP broth medium (Malt extract 3.00, Yeast extract 3.00, Peptone 5.00, Glucose 10.00 g/l, pH = 6.2;17, and incubated at 25 °C for 14 day under static conditions. Then the grown mycelium was separated by filtration using Whatman filter paper no.1. The culture filtrate was taken and filtered using 0.45 µm sterile cellulose nitrate membrane filter (Sartorius; Germany) and used for iron nanoparticles production extracellularly as following; 10 ml of culture filtrate was inoculated in 90 ml FeSo4.7 H2O solution in a final salt concentration of 0.1 mM, pH = 5.7. The mixture was incubated at room temperature at 150 rpm for 2 h. Iron nanoparticles production was detected by observing color change of sample and measuring its UV–Vis spectrum in comparison to salt free control in the range of 200–800 nm (JASCO V630). The UV–Vis spectrum of control sample containing zero salt concentration was used as the basal line for detecting the zero valent iron nanoparticle peaks. This test was carried out in triplicate manner.
Behavior of biosynthesis of iron nanoparticles over time was monitored by incubating the reaction mixture for different time periods (30 min, 2 h, 6 h and 24 h) and measuring UV-Vis spectrum of samples in a range of 200–800 nm to track the appearance of iron nanoparticles beak.
Size of synthesized iron nanoparticles was initially checked by Dynamic light scattering (DLS) technique using nano-zeta sizer (Malvern ZS-Nano, Scattering angle of 90). The dynamic light scattering technique is ideal for the measurement of the size of colloids and nanoparticles. The principle of that technique is that the fine particles and molecules are in constant Brownian motion, which its speed depends on their size. This speed can be monitored using the speckle pattern produced by illuminating the particles with a laser. The scattering intensity at a specific angle fluctuating with time is detected using a sensitive avalanche photodiode detector (APD). The intensity changes are analyzed with a digital autocorrelator which generates a correlation. This curve can be analyzed to give the size and the size distribution.
Additionally, the estimated size range was further confirmed by employing Transmission Electron Microscopy (JEM 2100 HRT, Japan, 200 kV).
Vibrating sample magnetometer was employed to evaluate the magnetic behavior of synthesized iron nanoparticles (iron NPs) at room temperature. Measurement was carried out with a magnetic field up to 20 kG. The vibrating sample magnetometer (VSM) is one of the most successful operation methods of a magnetometer. VSM measurements are unaffected with mass and size of sample.
Nature of compounds involved in the production and capping of synthesized iron nanoparticles have been checked by estimating the type of functional groups and bonds found in both the crude culture filtrate and biosynthesized iron nanoparticles. Spectra were detected using (Alpha II, Bruker, USA) at range of 4000–400 cm−1 and the measurements were recorded as the percent Transmission (% T).
Levels of some vital factors that can affect the nano iron synthesis have been optimized employing response surface methodology method “using Box-Behnken design”. Starting salt concentration, volume of added culture filtrate and pH were the tested factors. These three factors have been monitored at three levels, high (+ 1), low (− 1), and intermediate (0) as shown in Table 1.
The experiment was performed in fifteen trials, Table 2, three of them are central points to avoid error. Size of obtained particles was measured by DLS technique using nano-zeta sizer (Malvern ZS-Nano, with a scattering angle of 90) and that considered as the main monitored response. Results were fitted with a second-order polynomial equation which its general form is:
Y: predicted response, β0: the intercept term, βi: the linear coefficient, βij: the quadratic coefficient, βii: the interaction coefficient, and XiXj: the independent variables18.
Statistical analysis was performed on obtained data using software package ‘Design Expert’ software (Version 7.0). Accuracy of polynomial model equation was evaluated by checking the values of R2 and adjusted R2. Three-dimensional response surface plots have been used to express the fitted polynomial equation. Optimizing the levels of tested factors was employed using the Design Expert’s numerical optimization tool in a trail to yield the best response (particle size). The goal of optimization process was to minimize the size of produced iron nanoparticles “minimize the response”.
One of the predicted solutions that have been estimated by the numerical optimization tool for the best response results has been selected and tested experimentally. The percentage of deviation between the predicted and experimentally recorded values has been calculated to check and proof the accuracy of the obtained model.
Effectiveness of optimization process was evaluated by monitoring the amount and the characters of produced iron nano particles before and after applying the optimized conditions.
Size and surface charge of synthesized iron nanoparticles have been measured before and after optimization process using nano-zeta sizer (Malvern ZS-Nano, Scattering angle of 90).
Shape and topography of synthesized iron nanoparticles before and after optimization study have been monitored using scanning electron microscopy (JEOL, JSM-6360LA, Japan).
Percentage of iron in prepared samples before and after optimization process was checked using EDX along with Scanning Electron Microscopy (JEOL, JSM-6360LA, Japan Scanning Electron Microscope coupled with an Energy Dispersive X-ray spectrometer).
Quantity of produced iron nanoparticles under optimized and nonoptimized conditions was assessed by measuring the weight of dried nanoparticles. 100 ml of prepared nanoparticle solution was dried on oven at 60 °C for 72 h, then dry weight of produced nanoparticles was measured. The test was carried out in duplicated manner and a zero-salt solution “reaction mixture with zero salt concentration” has been used as a control.
Decolorization of methylene blue by the biosynthesized iron nanoparticles in presence of H2O2 has been tested. One milligram of iron nanoparticles was added to ten ml methylene blue solution (0.1 mg/l) and one ml of 10% H2O2. Decolorization of dye in absence of iron nanoparticles also in absence of both H2O2 and iron nanoparticles has also been evaluated. The reaction mixture was incubated at room temperature for 3 and 6 h. Absorbance of samples was measured at 665 nm to track methylene blue decolorization19. Test was performed in duplicated manner and percent of decolorization was calculated as following:
A0 = initial absorbance (at zero time), At = absorbance after each time interval19,20.
Antifungal activity of the biosynthesized iron nanoparticles against four fungal plant pathogens (Fusarium solani, Fusarium semitectum, Alternaria solani and Sclerotium rolfsii) has been estimated in a concentration of 5 and 50 ppm. The test was performed using percent inhibition of mycelial growth method (PIMG)21,22. Iron nanoparticles have been added to warm Czapek-Dox’s agar medium (Sucrose 30.00, NaNO3 3.00, KH2PO4 1.00, MgSO4 0.50, KCl 0.50, FeSO4 0.01, Agar 20 g/l, pH = 7.0;23), with the tested concentrations, mixed well and poured on sterilized petri dishes. After solidification, 7-day old fungal plugs (0.8 cm) were inoculated on the plates and incubated at 25 °C for 5 days. The test was carried out in duplicated manner and Czapek-Dox’s agar plates with zero nanoparticles concentration were used as control. The result was recorded by measuring the growth diameter and calculating of PIMG as following:
Gc: Growth diameter in control plate. Gt: Growth diameter in plates inoculated with nanoparticle.
The comparisons between two groups were performed by two groups t-Test analysis at 95% confidence interval using Sigma plot statistical package version 12.5. Standard deviation for the mean values was estimated. Statistical analysis for DOE optimization study was carried out by ‘Design Expert’ software package Version 7.0.
Sequencing of ITS4 region for the obtained isolate, revealed that; this isolate shows 99.45% similarity with different strains belonging to the following fungal species; Aspergillus flavipes, Aspergillus micronesiensis, Aspergillus neoflavipes. The phylogenetic tree analysis, Fig. 1, showed that; this isolate is found in a common joining with Aspergillus flavipes clusters, suggesting a new strain of Aspergillus flavipes. This identification was confirmed by checking the growth, macroscopic and microscopic characters of the investigated isolate. The investigated isolate on Czapek’s Dox media gives white to buff-yellowish colonies with off-white spores and reverse with brown shades producing no soluble pigments. These characters agree with characters described for A. flavipes24,25,26. On Malt Extract Agar (MEA) medium it gives similar characters. It showed no growth at 40 °C as described by Sklenář et al.27. Microscopical examination showed that, it forms spathulate conidial head. Swollen Hülle cells also have been observed and discrete masses of Hülle cells has been detected which agrees with microscopical features described for A. flavipes25,28,29, Fig. 2. According to the previous morphological characters the identification of the investigated isolate as A. flavipes has been confirmed. The previous growth and morphological description excludes the identification probabilities of Aspergillus neoflavipes and Aspergillus micronesiensis (A. frequens; A. micronesiensis synonymous) as Aspergillus neoflavipes shows bright yellow colonies on MEA28,30 and able to grow at 40 °C27, on the other hand, Aspergillus micronesiensis forms globose radiating conidial head and its colonies give soluble orange brown pigment29 and these characters do not match with the morphological and growth character of the investigated isolate. Thus, the obtained sequence was submitted in GenBank as Aspergillus flavipes isolate RMMB and released in GenBank under the accession number of MN956655.1.
Phylogenetic tree analysis of obtained ITS4 sequence (NCBI); the analyzed sequence is highlighted.
Aspergillus flavipes MN956655. (a); colony on Czapek’s Dox medium, (b); reverse of plate, (c); conidial head, (d); Hülle cells.
Upon addition of the fungal culture filtrate, the reaction mixture showed a color change from clear pale yellow to blackish brown which represents an initial indication for the reduction of Fe+2 ions by the culture filtrate and the production of iron nanoparticles, Fig. 3. Moreover, UV–Vis spectrum showed the formation an absorption beak at the triplicated samples at 250–268 nm, Fig. 4.
(a) Color change due to iron nano particles production, (b) control with zero salt concentration.
UV–Vis spectrum showing the formation of the nano iron characteristic beak in replicated samples.
Monitoring of iron nanoparticles’ synthesis over different time intervals showed that, the beak of iron nanoparticles has been detected just after 30 min from the beginning of the reaction and the absorption value increased with time to reach its best reading after 6 h. No shift has been observed in beak position with time (all nearly at 330 nm), Fig. 5.
UV–Vis spectrum for tracking nano iron synthesis over different time intervals.
The Dynamic light scattering analysis showed that the estimated size of the produced iron nanoparticles ranges between 32.7 and 47.6 nm, Fig. 6, while TEM micrographs have recorded their size in range of 19.45–29.75 nm, Fig. 7.
DLS measures showing size distribution of iron nanoparticles synthesized by Aspergillus flavipes MN956655.1.
TEM image of Aspergillus flavipes MN956655.1 mediated iron nanoparticles showing the electron diffraction pattern on the top.
The magnetic behavior of synthesized iron nanoparticles was assessed by Vibrating Sample Magnetometer (VSM). The obtained magnetization curve showed the formation of a hysteresis loop, Fig. 8. The estimated coercivity value (Hc) was 28.598 G (2.8 KA/m). The reported saturation magnetization value of the synthesized particles was 165.2 memu/g.
Magnetization curve of the biosynthesized iron nanoparticles showed the formation of a hysteresis loop.
FT-IR spectra of Aspergillus flavipes MN956655.1 culture filtrate and iron nanoparticles synthesized by it, Fig. 9, showed common bands at 3401, ~ 2960, 2937, 2880, ~ 1452, ~ 1400, 1660, ~ 1159, ~ 1109, 1200, ~ 1334 and ~ 1311 cm−1. On the other hand, FT-IR spectrum of biosynthesized iron nanoparticles showed the appearance of unique beaks in 400–600 cm−1 range and a further unique beak at 3734 cm−1.
Fourier transform infrared (FTIR) spectra of (a) Aspergillus flavipes MN956655.1culture filtrate, (b) iron nanoparticles synthesized by Aspergillus flavipes MN956655.1.
ANOVA analysis of the employed Box-Behnken model showed that the model p value was 0.0084, the model F-value was 10.98 and the Lack of Fit was not significant (The “Lack of Fit F-value” was 0.43), Table 3. Value of the model Correlation coefficient (R2) equals 0.95, while the adjusted R2 value equals 0.87, Table 4. According to the statistical analysis, B, BC, A2 and C2 are significant model terms where P < 0.05. The second-order polynomial equation estimated by the model regression analysis was as following:
where A, B and C are the coded factors of salt concentration, culture filtrate volume and pH respectively. The main effect of these factors and the interaction among them are visualized by Pareto chart, Fig. 10, the perturbation and the three-dimensional response plots. Perturbation plot, Fig. 11, shows the main effect of tested factors on the particle size. It has been observed that the volume of added culture filtrate has the major effect, where the increase in the added volume leads to a reduction in size of the produced iron nanoparticles.
Pareto chart showing the effect of tested factors on particle size of the produced iron nanoparticles according to Box-Behnken design analysis.
Perturbation plot showing the main effect of A = Salt conc., B = Culture filtrate volume, C = pH on size of produced iron nano particles.
The 3D response plots, Figs. 12, 13 and 14, were displayed by using two factors and keeping the third at the zero coded level (its intermediate value) to illustrate both the main and the interactive behavior of tested factors. They revealed that there is a significant mutual interaction between the volume of added culture filtrate and the pH value. The smallest particle size which can be obtained was calculated by the aid of Design Expert’s numerical optimization tool which predicts to be 42.9 nm when salt concentration, volume of added culture filtrate and pH equal 0.772 mM, 19.97 ml and 4.31 respectively, Fig. 15.
3-D. Response surface plot showing the interaction between the filtrate volume and salt conc. and its effect on the produced particle size.
3-D. Response surface plot showing the interaction between the pH and salt conc. and its effect on the produced particle size.
3-D. Response surface plot showing the interaction between the pH and filtrate volume and its effect on the produced particle size.
A predicted solution estimated by Box-Behnken design numerical optimization that used in validation of model to yield the smallest particle size.
The previous predicted solution for yielding the best particle size was used as a check point for model validation by testing the approximated values of its levels experimentally and comparing the actually obtained result with the predicted one. It has been found that the produced iron nanoparticles have recorded a particle size of 41.5 nm, giving a deviation of 3.3% from the model predicted value, Table 5.
Characters and amount of produced iron nanoparticles have been checked before and after applying the optimized conditions, Table 6, to evaluate the efficiency of the performed optimization study.
Concerning the particle size, nanoparticles have recorded a size of 48.2 ± 8.9 nm before applying the optimized conditions and a size of 41.5 ± 7.1 nm after applying the optimized levels (t-test P-Value = 0.308).
Similar response has been observed for surface charge, where zeta potential analysis revealed that the particles have a charge of − 33.5 ± 5.3 and − 33.4 ± 6.5 before and after applying the optimization levels respectively (t-test P-Value = 0.496), Fig. 16.
Size (a) & surface charge (c) of synthesized iron nanoparticles before employing the optimized conditions. Size (b) & surface charge (d) of synthesized iron nanoparticles after employing the optimized conditions.
Regarding the morphology of the produced iron nanoparticles, SEM results showed that the truncated tetrahedron shape is the most recorder shape among the nanoparticles before optimization study and the tetrahedron shape is the common shape after applying the optimized conditions, Fig. 17.
SEM micrographs showing morphology of biosynthesized iron nano particles before (a) and after (b) optimization study.
Checking the quantity of iron in the prepared nanoparticles before and after employing the optimized conditions has been performed using EDX analysis. EDX spectra, Fig. 18, revealed the presence of absorbance peaks at approximately 1 and 7 keV which are correlated to iron nanocrystal. Other major beaks have been reported for C, N, O and S. Iron percentage in the prepared nanoparticles was 0.97% and 3.87% before and after applying the optimized levels respectively, Table 7.
EDX Spectra showing iron percentage in prepared samples before (a) and after (b) employing optimization process.
Concerning the yield, the amount of the produced iron nanoparticles was 4.2 ± 1.6 mg/100 ml before applying the optimized conditions and that amount has increased to 19 ± 2 mg/100 ml after the optimization process (t-test P-Value = 0.0143).
Figure 19 summarizes the variability in particle size, surface charge, amount of iron nanoparticles and the variability in their iron percent before and after employing the optimization process.
Effect of optimization process on characters and quantity of produced iron nanoparticles.
Efficacy of the biosynthesized iron nanoparticles in methylene blue decolorization was evaluated in the presence of H2O2. The obtained results showed that, there is an obvious decrease in absorbance of methylene blue solution treated with iron nanoparticles in comparison to solution treated only with H2O2 which reflects a decolorization ability of the tested nanoparticles. It has been found that, decolorization percentage reaches 57 ± 4.3% after 6 h in case of iron nanoparticles, but solution treated only with H2O2 has recorded 23.4 ± 0.7 decolorization percentage. Methylene blue solution without H2O2 or iron nanoparticles treatments showed zero decolorization percentage after the tested periods, Fig. 20.
Methylene blue decolorization ability of biosynthesized iron nanoparticles.
Testing of antifungal activity showed that the use of iron nanoparticles with 50 ppm concentration has exerted a detectable inhibition especially against Alternaria solani (18.6 ± 0.8 inhibition percent), Table 8.
In this study, a new Aspergillus flavipes isolate has been obtained from soil sediment of Avicennia marina (Forssk.) Vierh. growing on Red Sea coast at Ras Mohammad—South Sinai—Egypt. It has been identified according to both its ITS4 sequence analysis and its growth, macroscopic and microscopic characters. Its ITS4 sequence was submitted in GenBank as Aspergillus flavipes isolate RMMB and released in GenBank under the accession number of MN956655.1. Production of iron nanoparticles by this isolate has been detected upon the addition of its culture filtrate to the FeSo4.7 H2O solution, where a color change has been observed from the clear pale yellow to blackish brown which represents an indication for the reduction of Fe+2 ions to their zero state. Formation of iron nanoparticles has also been tracked by scanning UV–Vis spectrum of prepared samples. UV–Vis spectrum showed the formation of an absorption beak at 250–268 nm which agree with the optical properties of Surface Plasmon Resonance of the iron nanoparticles and fits the described maximum absorption range characteristic for iron nanoparticles (250–350 nm)4. Tracking of the iron nanoparticles production over different time intervals using UV–Vis spectroscopy showed the formation of its characteristic beak just after 30 min. after initiating the reaction; and the position of such beak didn’t shift over the tested time intervals, which can indicate a stabilization in particle size for the formed iron nanoparticles over the tested periods.
The most important parameter that must be considered in synthesis of nanoparticles is their size range where it is significant in determining the effectiveness of such particles in the different application fields31. Thus, size of the produced iron nanoparticles has been estimated using dynamic light scattering (DLS) technique and transmission electron spectroscopy (TEM). Dynamic light scattering results has recorded the particle size in the range of 32.7—47.6 nm; and the TEM micrographs have estimated the size in the range of 19.45—29.75 nm. That recorded variability between the DLS and TEM measures is within the accepted range which can be explained by the presence of the nanoparticles during the DLS analysis as a suspended particles in a solution. Hence, they may be surrounded by other ions which can interfere with their exact size32. Xiao et al. utilized tea extract in green synthesis of iron nanoparticles but the synthesized particles have recorded size range of 75–100 nm20. Also, iron nanoparticles synthesized by endophytic Penicillium oxalicum have showed a relatively large size (140–170 nm)19. Thus, utilizing Aspergillus flavipes isolate RMMB in iron nanoparticles represents an enhanced biosynthesis method in terms of the particle size.
Concerning the magnetic behavior of the biosynthesized iron nanoparticles, it has been observed that; their magnetization curve showed the formation of a hysteresis loop which indicates a ferromagnetic behavior for the synthesized iron nanoparticles. Moreover, the estimated coercivity value (Hc) was 28.598 G (2.8 KA/m). The magnetic materials with coercivity less than 1 kA/m are considered as magnetically soft and the materials with coercivity higher than 10 kA/m are considered as hard magnetic materials33. The estimated coercivity value fits the range in between soft and hard ferromagnetic materials which reflects the semihard ferromagnetic behavior of synthesized iron nanoparticles. The reported saturation magnetization value of the synthesized particles was 165.2 memu/g. This value is higher than the magnetization value reported by Kheshtzar et al. for zero-valent iron nanoparticles synthesized by extracts of green tea leaves which was around 80 memu/g34. Also, that recorded value is better than the magnetization value reported for iron oxide nanoparticles coated with green tea polyphenols and those synthesized by tannin extract from Acacia mearnsii where their values were 61 memu/g35 and 3.2 memu/g36 respectively. These reduced values were explained by the diamagnetic effect of capping biological materials and amorphous state of the prepared iron nanoparticles34. Thus, the obtained results reveal an enhanced magnetic property for the biosynthesized iron nanoparticles compared to other green synthesized ones giving them more suitable characteristics for the remediation applications as the magnetic manipulation is required.
FT-IR spectroscopy was employed to identify and characterize the functional groups associated with the biosynthesis and stabilization of iron nanoparticles. Monitoring of such functional groups can be used in interpretation of the chemical nature of biomolecules that may be involved in synthesis and capping of biosynthesized nanoparticles. FT-IR spectra of Aspergillus flavipes MN956655.1 culture filtrate and iron nanoparticles synthesized by it showed a significant common band at 3401 cm−1 which corresponds to bonded O–H stretch31,37,38. Also, they revealed other common bands at ~ 2960, 2937, 2880, ~ 1452 and ~ 1400 cm−1 that are related to C–H and C–H2 stretching4,31,37,38,39. Another common band in 1660 cm−1 is observed which reflects aromatic combinations and C=C aromatic ring stretching vibration31,34,37,38. On the other hand, presence of bands in ~ 1159 and ~ 1109 cm−1 represents possible indication for C–O stretch for tertiary and secondary alcohol respectively37,38. Moreover, a band in 1200 cm−1 is detected which corresponds to phenolic C–H stretch34,37,38. All previous signals reveal possible involvement of flavonoids, phenolic4 and alcoholic compounds in synthesis and capping of iron nanoparticles. In addition to that, common bands have been observed in ~ 1334 and ~ 1311 cm−1 which may belong to aromatic C–N stretching34,37,38 and that indicates a possible role of the aromatic nitrogenous compounds in synthesis and capping process. FT-IR spectrum of biosynthesized iron nanoparticles showed the appearance of unique beaks in 400–600 cm−1 range which correlated to Fe–O stretching vibration mode31,34,40 and that confirming the formation of magnetite iron nanoparticles. A further unique beak has been observed at 3734 cm−1 which corresponds to nonbonded hydroxyl group O–H37,38 stretch that may be due to residual water which surrounds the nanoparticles.
An optimization study has been performed employing experimental design method to reveal how to employ the production process under the most efficient conditions to enhance the iron nanoparticles’ production process. Experimental design methods represent a competitive and more effective methods for optimization process than the traditional method ‘one factor at time’ as the experimental design methods not only illustrate the effects of tested factors and optimize their levels but also can estimate the interaction between them41,42. As illustrated from the previous studies; pH, the volume of added culture filtrate and the used metallic salt concentration are among the most important factors affecting the nanoparticle synthesis process. Hence these three factors have been selected for the optimization study using experimental design method. ANOVA analysis of the employed Box-Behnken model showed that, the model is significant where the model p value was 0.0084. Also, it has been found that the value of the model Correlation coefficient (R2) equals 0.95 and the adjusted R2 value equals 0.87, which reflects a good correlation and supports the significance of the model. Concerning the main effect of tested factors, the volume of added culture filtrate has recorded the major effect, where the increase in the added volume leads to a reduction in size of the produced iron nanoparticles. Visualization of the interactive behavior of tested factors using the 3D response plots revealed the presence of a significant mutual interaction between the volume of added culture filtrate and the pH value. The model has predicted that, the smallest particle size which can be obtained is 42.9 nm when salt concentration, volume of added culture filtrate and pH equal 0.772 mM, 19.97 ml and 4.31 respectively. This prediction was tested experimentally for model validation, and it has been found that; employing the production process under the prementioned levels yields iron nanoparticles with a size of 41.5 nm giving a deviation of 3.3% from the model predicted value and that represents an acceptable error range which recommends the validation of the employed model. Similar results have been reported by Kheshtzar et al., where they have recorded a significant role for the volume of green tea extract used in biosynthesis of iron nanoparticles after employing an optimization study using a response surface methodology design34. Also, Singh et al. have optimized biosynthesis of iron oxide nanoparticles by leaf extract of Coriandrum sativum L. (cilantro) utilizing response surface methodology and they have estimated that, the volume of added leaf extract is a significant factor in synthesis process. However, their optimization study failed to reduce the size of the produce nanoparticle below 161 nm43.
The efficiency of the performed optimization study was evaluated by comparing the size, shape, amount of the produced iron nanoparticles before and after employing the production process under the optimized conditions. conditions. Concerning the particle size, applying the optimized levels has led to a slight reduction in particle size. However, this size reduction was found to be statistically non-significant (t-test P-Value = 0.308). A similar response has been observed for surface charge. The biosynthesized iron nanoparticles have recorded a negative surface charge which can support their application in bioremediation process where they can attract and absorb positively cationic dyes. Moreover, the estimated value of surface charge (− 33.4 ± 6.5) can be considered high enough to maintain a reasonable stability for the prepared nano solution over storage. This reported value is better than the surface charge of iron oxide nanoparticles synthesized by Coriandrum sativum leave extract even after employing the optimization process where they have recorded only a potential of − 19.543.
Regarding the morphology of the produced iron nanoparticles, SEM results showed a clear variability in shape and topographic characters before and after applying the optimized conditions. The most common shape that has been observed among the nanoparticles before optimization study is the truncated tetrahedron while the most recorded shape after applying the optimized conditions is the tetrahedron which can be correlated to the increased salt concentration used in the production process. Similar behavior was reported by Zheng et al. during the preparation of gold nanoparticles, where the increase in gold precursor amount stimulated the formation of tetrahedron particles rather than the truncated tetrahedron shape44.
EDX analysis was performed to evaluate the elemental composition of prepared samples and check the quantity of iron in them before and after employing the optimized conditions. EDX spectra revealed the presence of absorbance peaks associated with surface plasmon resonance of iron nanocrystal at approximately 1 and 7 keV6,45. In addition to that, an enhanced iron percent has been obviously detected after applying the optimized levels where it has been increased from 0.97% to 3.87% (increased fourfold). Other major beaks have been reported for C, N and O that may be correlated to the biomolecules that involved in capping of iron nanoparticles, as discussed in results of FTIR analysis, while S may be derived from FeSo4 salt used as a precursor in synthesis process.
Regarding to the yield, it has been found that the amount of produced iron nanoparticles was significantly increased after employing the production process with the optimized conditions, where it increased from 4.2 ± 1.6 mg/100 ml to 19 ± 2 mg/100 ml (t-test P-Value = 0.0143; fourfold and half increase).
Hence it can be concluded that; the performed optimization process has greatly affected the morphology of the produced iron nanoparticles where it stimulated the formation of particles in tetrahedron shape without increasing the particle size or changing the value of their surface charge. Moreover, the optimized conditions have increased the yield of the produced iron nanoparticles nearly by fourfold.
Effectiveness of the produced magnetic iron nanoparticles in decolorization of methylene blue has been estimated. Use of iron nanomaterials in water treatment processes represent a highly significant technique compared to the other nanomaterials mediated approaches such as adsorption processes (using nano clays and ceramics), use of nanofiltration techniques, use of carbon nanotube and nano-catalyst (for example: titanium dioxide). Iron nano particles show some important characters making them an efficient tool for this purpose as they are less toxic, biodegradable and with high reactivity and large surface area. Additionally, they have a magnetic affinity and dual redox properties on water. Moreover, the precursors used in synthesis of iron nanoparticles are widely available and have low cost46. Zero-valent iron nanoparticles employ high adsorption ability, strong reducibility and good catalytic activity; thus, they alone can act as an efficient reducing agent in bioremediation processes. However, their efficiency can be enhanced in presence of oxygen compounds such as hydrogen peroxide, where in this case they produce reactive oxygen species efficiently that play a role in the degradation of organic contaminants34. Iron nanoparticles synthesized in this study has recorded good decolorization percent for methylene blue in presence of H2O2 where they have yielded 57 ± 4.3 decolorization percent after 6 h. Similar results have been recorded for iron oxide nanoparticles which yielded 55.25% degradation for methylene blue47 and these recorded decolorization values are better than that recorded for silver nanoparticles synthesized by cyanobacteria where they showed only 18% decolorization for methylene blue after 4 h48. Although the biosynthesized iron nanoparticles have been tested in this study with low concentration (0.1 mg/ml), they have yielded a good decolorization percent “46.8 ± 4%” for methylene blue after 3 h comparing to the efficiency of zero valent iron nanoparticles biosynthesized by Kheshtzar et al. in decolorization of methyl orange where they have yielded a similar decolorization percent after 2 h but by using higher nanoparticle concentration “1 mg/ml”34.
Finally, the antifungal activity of the biosynthesized iron nanoparticles has been estimated against different fungal plant pathogens. It has been found that, these nanoparticles have exerted a detectable inhibition against Alternaria solani (18.6 ± 0.8 inhibition percent) when tested with only 50 ppm concentration. Ali et al. have recorded a relatively similar inhibition percent (23.9 ± 0.0) against A. alternata when it was treated with higher nano iron concentration (100 ppm/ “0.1 mg/ml”). On the other hand, iron oxide nanoparticles showed lower antifungal behavior against Alternaria alternata when tested by the same concentration49. Also, Parveen et al. have recorded a lower antifungal activity for iron oxide nanoparticles synthesized by tannic acid against Alternaria alternata by assessing their inhibition for spore germination, as 100 ppm concentration (0.1 mg/ml) has yielded only 8.59% inhibition for spore germination50.
Magnetic iron nanoparticles have been synthesized by a green method using Aspergillus flavipes MN956655. This is the first study carried out on the biosynthesis of magnetic iron nanoparticles by Aspergillus flavipes. This investigated biosynthesis method yields magnetic iron nanoparticle with competitive characters compared to magnetic iron nanoparticles synthesized by plant extracts. The produced nanoparticles have recorded a good size range (32.7–47.6 nm) and a surface charge of − 33.5 ± 5.3 which can maintain a reasonable stability for the prepared nano solutions over storage. These particles showed a semihard ferromagnetic behavior and a magnetization value higher than that recorded for many biosynthesized iron oxide and zero valent iron nanoparticles which reflects less diamagnetic effect of capping biomaterials used in this synthesis process. Optimized conditions stimulated the formation of nanoparticles in tetrahedron shape rather than the truncated tetrahedron shape and efficiently increases the quantity of iron in prepared samples and the amount of produced iron nanoparticles to a fourfold. These biosynthesized iron nanoparticles showed good decolorization behavior for methylene blue. These findings can be utilized in solving one of the potential environmental issues as these nanoparticles can be used in decolorizing of dyes in water in a trial to treat industrial effluents and minimize their environmental hazards. Moreover, the produced iron nanoparticles have exerted a detectable antifungal activity against one of the most important plant pathogens “Alternaria solani” with only 50 ppm concentration.
All data generated during this study are included in this published article. Other raw data analyzed during the current study are available from the corresponding author on reasonable request.
Ameen, F., Alsamhary, K., Alabdullatif, J. A. & Al Nadhari, S. A review on metal-based nanoparticles and their toxicity to beneficial soil bacteria and fungi. Ecotoxicol. Environ. Saf. 213, 112027 (2021).
Qamar, S.U.-R. & Ahmad, J. N. Nanoparticles: Mechanism of biosynthesis using plant extracts, bacteria, fungi, and their applications. J. Mol. Liq. 334, 116040 (2021).
Fahmy, H. M. et al. Review of Green Methods of Iron Nanoparticles Synthesis and Applications. BioNanoSci. 8, 491–503 (2018).
Sadhasivam, S., Vinayagam, V. & Balasubramaniyan, M. Recent advancement in biogenic synthesis of iron nanoparticles. J. Mol. Struct. 1217, 128372 (2020).
Pattanayak, D. S., Pal, D., Thakur, C., Kumar, S. & Devnani, G. L. Bio-synthesis of iron nanoparticles for environmental remediation: Status till date. Mater. Today 44, 3150–3155 (2021).
Mahanty, S. et al. Green synthesis of iron oxide nanoparticles mediated by filamentous fungi isolated from Sundarban Mangrove Ecosystem, India. BioNanoScience 9, 637–651 (2019).
Mahanty, S. et al. Mycosynthesis of iron oxide nanoparticles using manglicolous fungi isolated from Indian sundarbans and its application for the treatment of chromium containing solution: Synthesis, adsorption isotherm, kinetics and thermodynamics study. Environ. Nanotechnol. Monit. Manag. 12, 100276 (2019).
Anderson, M. J. & Whitcomb, P. J. Screening Process Factors in the Presence of Interactions. Stat-Ease, Inc. (https://www.statease.com/pubs/aqc2004.pdf) (2004).
Gorbounov, M., Taylor, J., Petrovic, B. & Soltani, S. M. To DoE or not to DoE? A technical review on & roadmap for optimisation of carbonaceous adsorbents and adsorption processes. S. Afr. J. Chem. Eng. 41, 111–128 (2022).
Madondo, N. I. & Chetty, M. Anaerobic co-digestion of sewage sludge and bio-based glycerol: Optimisation of process variables using one-factor-at-a-time (OFAT) and Box-Behnken Design (BBD) techniques. S. Afr. J. Chem. Eng. 40, 87–99 (2022).
Luiz, M. T. et al. Design of experiments (DoE) to develop and to optimize nanoparticles as drug delivery systems. Eur. J. Pharm. Biopharm. 165, 127–148 (2021).
Rakić, T., Jančić-Stojanović, B., Malenović, A., Ivanović, D. & Medenica, M. Demasking large dummy effects approach in revealing important interactions in Plackett-Burman experimental design.J. Chem.26, 518–525 (2012).
Waksman, S. A. A method for counting the number of fungi in the soil. J. Bacteriol. 7, 339–341 (1922).
Kumar, PKR, Hemanth, G., Niharika, PS & Kolli, SK Isolation and identification of soil mycoflora in agricultural fields at Tekkali Mandal in Srikakulam District.Int.J. Adv.Pharm.Biol.Chem.4, 484–490 (2015).
Doyle, J. J. & Doyle, J. L. A rapid DNA isolation procedure for small quantities of fresh leaf tissue. Phytochem. Bull. 19, 11–15 (1987).
Cullings, K. W. Design and testing of a plant-specific PCR primer for ecological and evolutionary studies. Mol. Ecol. 1, 233–240 (1992).
Wickerham, L.J. Taxonomy of Yeasts. Technical Bulletin U.S. Department of Agriculture no.1029 (1951).
Jose, P. A., Sivakala, K. K. & Jebakumar, S. R. D. Formulation and statistical optimization of culture medium for improved production of antimicrobial compound by Streptomyces sp. JAJ06. Int. J. Microbiol. 2013, 526260 (2013).
Mathur, P., Saini, S., Paul, E., Sharma, C. & Mehtani, P. Endophytic fungi mediated synthesis of iron nanoparticles: Characterization and application in methylene blue decolorization. Curr. Res. Green Sustain. Chem. 4, 100053 (2021).
Xiao, C., Li, H., Zhao, Y., Zhang, X. & Wang, X. Green synthesis of iron nanoparticle by tea extract (polyphenols) and its selective removal of cationic dyes. J. Environ. Manage. 275, 111262 (2020).
Imtiaj, A., Jayasinghe, C., Lee, G. W. & Lee, T. S. Antibacterial and Antifungal Activities of Stereum ostrea ‘an Inedible Wild Mushroom’. Mycobiology 35, 210–214 (2007).
Boonsang, N., Dethoup, T., Singburaudom, N., Gomes, N. G. M. & Kijjoa, A. In vitro antifungal activity screening of crude extracts of soil fungi against plant pathogenic fungi. J. Biopest. 7, 156–166 (2014).
Raper, K. P. & Fennell, D. I. The Genus Aspergillus 36 (Williams and Wilkins, 1965).
Kebeish, R. M. & El-Sayed, A. S. Morphological and molecular characterization of L-methioninase producing Aspergillus species. Afr. J. Biotech. 11(87), 15280–15290 (2012).
Raper, K. B. & Fennel, D. I. The Genus Aspergillus 558–561 (Williams and Wilkins, 1965).
Muntanjola-Cvetkovic, M. & Vukic, V. V. Influence of light on hülle cell and aleuriospore formation in Aspergillus. Trans. Br. Mycol. Soc. 58(1), 67–72 (1972).
Sklenář, F. et al. Re-examination of species limits in Aspergillus section Flavipedes using advanced species delimitation methods and description of four new species. Stud. Mycol. 99, 100120 (2021).
Abdel-Azeem, A. M. et al. The Egyptian Ascomycota 1: Genus Aspergillus. Microb. Biosyst. 5(1), 61–99. https://doi.org/10.21608/MB.2020.100044 (2020).
Visagie, C. M. et al. Aspergillus, Penicillium and Talaromyces isolated from house dust samples collected around the world. Stud. Mycol. 78, 63–139 (2014).
Arzanlou, M., Samadi, R., Frisvad, J. C., Houbraken, J. & Ghosta, Y. Two novel Aspergillus species from hypersaline soils of the National Park of Lake Urmia, Iran. Mycol. Progr. https://doi.org/10.1007/s11557-016-1230-8 (2016).
Bettencourt, G.M.-D.-F., Degenhardt, J., Torres, L. A. Z., Tanobe, V.O.D.-A. & Soccol, C. R. Green biosynthesis of single and bimetallic nanoparticles of iron and manganese using bacterial auxin complex to act as plant bio-fertilizer. Biocatal. Agric. Biotechnol. 30, 101822 (2020).
Fatemi, M., Mollania, N., Momeni-Moghaddam, M. & Sadeghifar, F. Extracellular biosynthesis of magnetic iron oxide nanoparticles by Bacillus cereus strain HMH1: Characterization and in vitro cytotoxicity analysis on MCF-7 and 3T3 cell lines. J. Biotechnol. 270, 1–11 (2018).
Mehboob, N. Hysteresis Properties of Soft Magnetic Materials (2012). Dissertation for Doctor of Science academic degree. 091 411 (2018).
Kheshtzar, R. et al. Response surface methodology and reaction optimization to product zero-valent iron nanoparticles for organic pollutant remediation. Biocatal. Agric. Biotechnol. 21, 101329 (2019).
Singh, K. K., Senapati, K. K. & Sarma, K. C. Synthesis of superparamagnetic Fe3O4 nanoparticles coated with green tea polyphenols and their use for removal of dye pollutant from aqueous solution. J. Environ. Chem. Eng. 5, 2214–2221 (2017).
Khan, M. Y. et al. Green chemistry preparation of superparamagnetic nanoparticles containing Fe3O4 cores in biochar. J. Anal. Appl. Pyrol. 116, 42–48 (2015).
Coates, J. Interpretation of infrared spectra: A practical approach. In Encyclopedia of Analytical Chemistry (ed. Meyers, R. A.) 10815–10837 (Wiley, 2000).
Nandiyanto, A. B. D., Oktiani, R. & Ragadhita, R. How to read and interpret FTIR spectroscope of organic material. Indonesian J. Sci. Technol. 4, 97–118 (2019).
Periakaruppan, R. et al. Utilization of tea resources with the production of superparamagnetic biogenic iron oxide nanoparticles and an assessment of their antioxidant activities. J. Clean. Prod. 278, 123962 (2021).
Zhang, Q. et al. Green synthesis of magnetite nanoparticle and its regulatory effect on fermentative hydrogen production from lignocellulosic hydrolysate by Klebsiella sp. Int. J. Hydrog. Energy 46, 20413–20424 (2021).
Baadhe, R. R., Mekala, N. K., Parcha, S. R. & Devi, Y. P. Optimization of amorphadiene production in engineered yeast by response surface methodology. 3 Biotech 4, 317–324 (2014).
Bai, Y., Saren, G. & Huo, W. Response surface methodology (RSM) in evaluation of the vitamin C concentrations in microwave treated milk. J. Food Sci. Technol. 52, 4647–4651 (2015).
Singh, K., Chopra, D. S., Singh, D. & Singh, N. Optimization and ecofriendly synthesis of iron oxide nanoparticles as potential antioxidant. Arab. J. Chem. 13, 9034–9046 (2020).
Zheng, Y. et al. Seed-Mediated synthesis of gold tetrahedra in high purity and with tunable well-controlled sizes. Chem. Asian J. 9, 2635–2640 (2014).
Salem, D. M. S. A., Ismail, M. M. & Aly-Eldeen, M. A. Biogenic synthesis and antimicrobial potency of iron oxide (Fe3O4) nanoparticles using algae harvested from the Mediterranean Sea, Egypt. Egypt. J. Aquat. Res. 45, 197–204 (2019).
Puthukkara, P. A. R., Sunil Jose, T. & Dinooplal, S. Plant mediated synthesis of zero valent iron nanoparticles and its application in water treatment. J. Environ. Chem. Eng. 9, 104569 (2021).
Vinothkannan, M., Karthikeyan, C., Kumar, G. G., Kim, A. R. & Yoo, D. J. One-pot green synthesis of reduced graphene oxide (RGO)/Fe3O4 nanocomposites and its catalytic activity toward methylene blue dye degradation. Spectrochim. Acta Part A Mol. Biomol. Spectrosc. 136, 256–264 (2015).
Article ADS CAS Google Scholar
Keskin, N. O. S., Kılıç, N. K., Dönmez, G. & Tekinay, T. Green synthesis of silver nanoparticles using cyanobacteria and evaluation of their photocatalytic and antimicrobial activity. J. Nano Res. 40, 120–127 (2016).
Ali, M., Haroon, U., Khizar, M., Chaudhary, H. J. & Munis, M. F. H. Facile single step preparations of phyto-nanoparticles of iron in Calotropis procera leaf extract to evaluate their antifungal potential against Alternaria alternata. Curr. Plant Biol. 23, 100157 (2020).
Parveen, S. et al. Preparation, characterization and antifungal activity of iron oxide nanoparticles. Microb. Pathog. 115, 287–292 (2018).
Open access funding provided by The Science, Technology & Innovation Funding Authority (STDF) in cooperation with The Egyptian Knowledge Bank (EKB).
Botany and Microbiology Department, Faculty of Science, Helwan University, Helwan, Egypt
You can also search for this author in PubMed Google Scholar
All research items (Design of the work, analysis, interpretation of data, writing and discussion) have been performed by N.H.A. All sections of the manuscript are relevant to the study performed by N.H.A.
Correspondence to Nashwa H. Abdullah.
The author declares no competing interests.
Springer Nature remains neutral with regard to jurisdictional claims in published maps and institutional affiliations.
Open Access This article is licensed under a Creative Commons Attribution 4.0 International License, which permits use, sharing, adaptation, distribution and reproduction in any medium or format, as long as you give appropriate credit to the original author(s) and the source, provide a link to the Creative Commons licence, and indicate if changes were made. The images or other third party material in this article are included in the article's Creative Commons licence, unless indicated otherwise in a credit line to the material. If material is not included in the article's Creative Commons licence and your intended use is not permitted by statutory regulation or exceeds the permitted use, you will need to obtain permission directly from the copyright holder. To view a copy of this licence, visit http://creativecommons.org/licenses/by/4.0/.
Abdullah, N.H. Optimization of magnetic nano-iron production by Aspergillus flavipes MN956655.1 using response surface methodology and evaluation of their dye decolorizing and antifungal activities. Sci Rep 12, 21059 (2022). https://doi.org/10.1038/s41598-022-25339-3
DOI: https://doi.org/10.1038/s41598-022-25339-3
Anyone you share the following link with will be able to read this content:
Sorry, a shareable link is not currently available for this article.
Provided by the Springer Nature SharedIt content-sharing initiative
By submitting a comment you agree to abide by our Terms and Community Guidelines. If you find something abusive or that does not comply with our terms or guidelines please flag it as inappropriate.
Scientific Reports (Sci Rep) ISSN 2045-2322 (online)
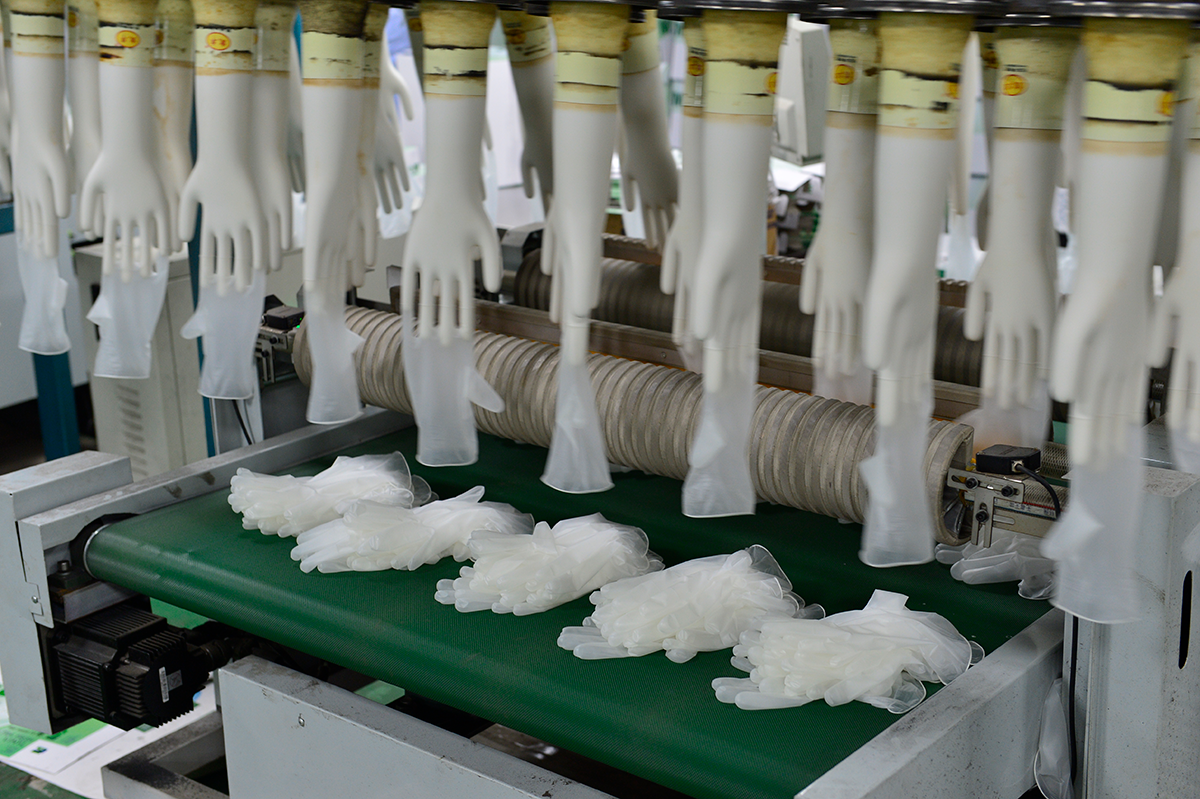
Decoloring Agent Sign up for the Nature Briefing: Translational Research newsletter — top stories in biotechnology, drug discovery and pharma.